Abstract
Despite mounting attention in recent years, health threats posed by antimicrobial resistance are not new. Antimicrobial resistance has dogged infectious disease treatment processes since the first modern antimicrobials were discovered.
An Evolutionary Arms Race
When recounting the history of medicine, few triumphs compare to the emergence and widespread use of antimicrobials. Alexander Fleming’s serendipitous discovery of penicillin on his petri dish1 brought about a new era in biomedicine. Suddenly, pathogens that had wreaked havoc for generations—spreading untold morbidity and mortality in their wake—were at the mercy of our chemical armamentarium. Seemingly overnight, infectious diseases receded before the ever-rising tide of antimicrobials, and optimistic observers in the United States and Europe predicted a swift and righteous victory over the scourge of infection.
Of course, such a victory was not achieved. Antibiotics are derived from the evolutionary arms race between microbes and their ecological competitors (fellow microbes, fungi, plants, and animals), and, as a result, the emergence of resistance is entirely predictable. As swiftly as we claimed new victories, microbes began evading our latest weapons, altering their cell walls, upregulating drug efflux systems, and dismantling and detoxifying our new wonder drugs.2,3
This story of innovation and setbacks is as old as time, familiar to anyone who works with pathogens, cares for patients, or develops new drugs. It begins with the early investigators and innovators who first recruited naturally occurring and synthetic chemicals in the fight against infectious diseases, and it continues toward an uncertain future.
Humans Harness Modern Antimicrobials
In 1907, Paul Ehrlich, a German physician-scientist, delivered a lecture to the Royal Institute of Public Health in London on the effect of aminophenylarsenic acid on trypanosomes (a type of single-cell parasite).4 In his address, Ehrlich detailed the synthesis of arsenicals, or arsenic-derived compounds, and their selective toxicity in treating sleeping sickness. In a moment of prescience, Ehrlich noted that while these medicines were remarkably successful in controlling the disease in mice, resistance to these compounds could be cultivated, passed on to new generations of trypanosomes, and maintained after sustained treatment. Despite this forewarning of the difficulties to come, the first battle in the war against infectious diseases had been won. The race to develop more of these compounds had begun.
It is perhaps the closest thing to a modern biomedical fairytale: Fleming’s plates of Staphylococci exposed to the air during laboratory work were contaminated by a mold and began to die.1 The 1929 discovery and isolation of penicillin ushered in a new era of biomedical research and discovery. Penicillin’s potency and limited side effects in humans—especially when compared to contemporaneous chemical antiseptics, such as carbolic acid—led to its immediate recognition as a potential topical and systemic treatment for pyogenic infections. Its use as a selective agent in bacterial culture media also allowed for the reliable isolation of penicillin-tolerant microorganisms for the purposes of diagnosis and scientific research.5
Early Uses of Penicillin
Unsurprisingly, Fleming’s work was recognized by antimicrobial researchers for its revolutionary potential. At Oxford, a team assembled by Howard Florey and Ernst Chain set out to isolate penicillin and assess its antimicrobial effects. In 1940, Florey’s team published its study of the efficacy of penicillin in vivo. The effect of this new wonder drug could not be understated: among mice infected with relevant human pathogens, all untreated animals succumbed to their infections within 10 days, but those treated with penicillin had dramatically improved survival rates.6 With the knowledge that penicillin was both efficacious and well tolerated in mice, Florey’s team set its sights on human trials.
In 1940, a 43-year-old police officer, Albert Alexander, was admitted to the Radcliffe Infirmary at Oxford for an infection of the face, scalp, and orbits. He was treated with first-generation sulfonamide antibacterials; however, over several months, his condition continued to worsen. On February 12, 1941, Florey’s team started an infusion of penicillin and saw rapid clinical improvement. Unfortunately, by the fifth day the supply of penicillin had been exhausted, and Alexander’s clinical status again began to decline. On March 15, 1941, Alexander succumbed to his infection. His autopsy indicated staphylococcal infection and osteomyelitis as the cause of death.7
The first use of Fleming’s wonder drug was a disappointment. Despite efficacy in mice, tolerability in humans, and transient improvement in the patient’s infection during treatment, there simply was not enough supply to meet the needs for effective, curative dosing and duration in a human patient. Scarcity—not lack of efficacy—led to the failure of the first therapeutic regimen of penicillin.
Florey’s team, however, was undeterred. In the same report published in 1941, Florey’s team detailed remarkable advances in the ability to purify, concentrate, and deploy penicillin at therapeutic doses. Florey’s team had determined that, after intravenous administration, penicillin was excreted in the patients’ urine. This penicillin could then be recovered, purified, and reinfused, momentarily overcoming the problem of scarcity. With the ability to essentially recycle penicillin and maintain bacteriostatic concentrations of penicillin in the blood, clinical outcomes improved, and patients began to be cured of penicillin-susceptible infections.7 While barriers to the widespread use of the drug—scarcity, access, and deployment—remained, the first true superweapon in the fight against infectious diseases had emerged.
Resistance Evolves
As soon as this new antimicrobial was discovered—and even before the successful treatment of patients at the Radcliffe Infirmary in London—researchers were beset with the problem of resistance. In a 1940 letter to the editor of Nature, Oxford biochemists Edward Abraham and Chain reported a startling discovery: an enzyme isolated from penicillin-insensitive Escherichia coli could break down penicillin and hamper its bacteriolytic functions.8 Researchers next sought to understand how resistance developed and whether the antimicrobials themselves played a role in their own inconsistent or waning efficacy. Milislav Demerec demonstrated that antibacterial resistance arose spontaneously in bacterial cultures as a function of random genetic mutations, although the mutations themselves were not a direct result of antibiotic exposure. However, exposure to antibiotics selected for resistant bacterial strains and allowed them to persist.9
Antimicrobials in the Modern Era
The decades following the implementation of penicillin saw incredible research and innovation in the field of antimicrobials.10 An escalating cycle of discovery, implementation, and emergence of resistance drove the development of new classes of antibacterials, including the modified beta-lactams, cephalosporins, fluoroquinolones, and aminoglycosides still used today (see Figure). Despite the emergence of resistance to new antibacterials, scientists and pharmaceutical companies were generally able to keep pace through the mid-20th century, deriving new compounds from natural products and modifying them to suit clinical needs.
Figure. Key Events in Antimicrobial Discovery and Resistance
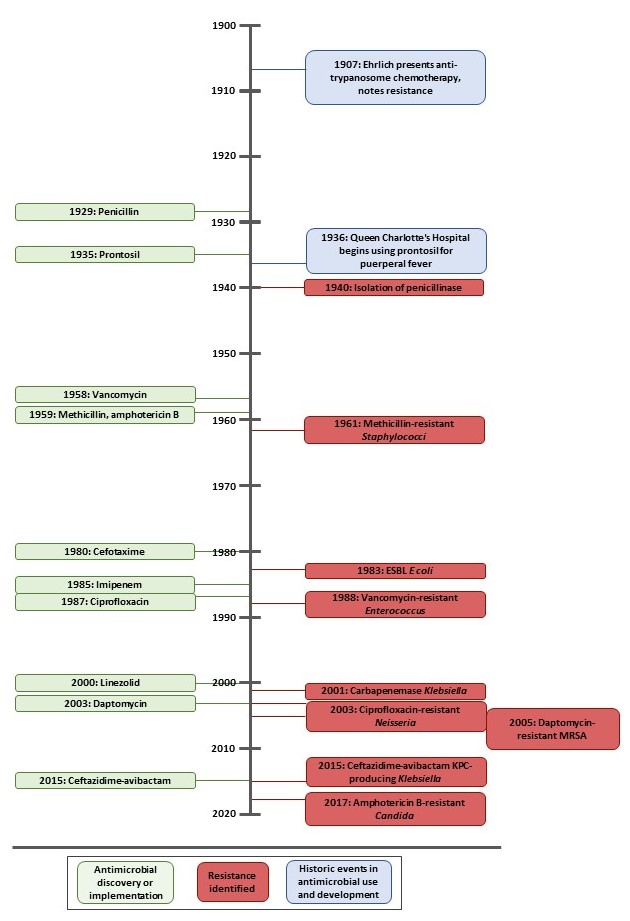
Data sources: Fleming A1; Erlich P4; Abraham EP, Chain E8; Colebrook L, Kenny M11; Little JS, Dedrick RM, Freeman KG, et al12; Schooley RT, Biswas B, Gill JJ, et al13; Barber M14; Knothe H, Shah P, Krcmery V, Antal M, Mitsuhashi S15; Leclercq R, Derlot E, Duval J, Courvalin P16; Yigit H, Queenan AM, Anderson GJ, et al17; Fenton KA, Ison C, Johnson AP, et al; GRASP collaboration18; Mangili A, Bica I, Snydman DR, Hamer DH19; Humphries RM, Yang S, Hemarajata P, et al20; Lockhart SR, Etienne KA, Vallabhaneni S, et al.21
Abbreviations: ESBL, extended spectrum beta-lactamase; E coli, Escherichia coli; MRSA, methicillin-resistant Staphylococcus aureus.
Against this backdrop of innovation and resistance, antimicrobials found another application: agriculture. With the rise of industrial farming, animals were kept in increasingly crowded and often unsanitary conditions, and antimicrobials were utilized prophylactically in livestock to increase growth rates and prevent illness.22,23 Unlike in the clinical setting, in agriculture the use of antimicrobials is not subject to the same oversight or guidelines for prescribing. The lack of consistent regulation permits wide variation in terms of the classes and concentrations of antimicrobials used in agriculture. Often, livestock are given subtherapeutic doses of antibacterials, which creates an environment of selective pressure that fosters emergence of resistance among the bacteria in the animals’ bodies.23 Despite early research indicating the potential for antimicrobial resistance to spread from bacteria in livestock to bacteria in human hosts,24 the use of clinically important antimicrobials in agriculture persists into the present, and demand for meat continues to rise. In 2021, an estimated 54% of the 11 million kilograms of antimicrobials sold for use in domestic agriculture in the United States belonged to the “medically important” category.25
Low- and middle-income countries face a disproportionate burden of antimicrobial resistance and associated deaths.
While widespread use of antimicrobials in both health care and agricultural settings created an environment for resistance to flourish, the discovery of new antibiotics slowed.26 Soon enough, antimicrobial drug development began to clash with the realities of an economic system predicated on supply and demand.27 As death rates from cancer and heart disease rose to replace deaths from infectious diseases, pharmaceutical companies faced slimmer economic margins for developing new anti-infectives. The rate of discovery of new antibiotics slowed, and those few specialized drugs that were developed to overcome antimicrobial resistance (eg, carbapenems, lipopeptides, oxazolidinones, novel tetracyclines, and novel beta-lactam/beta-lactamase inhibitor combinations) were expensive to use.28 With the latest antimicrobials often only available in highly resourced settings, low- and middle-income countries (LMICs) face a disproportionate burden of antimicrobial resistance and associated deaths. An antimicrobial resistance research group estimated that 1.27 million deaths globally in 2019, including over a million in LMICs, were attributable to antimicrobial-resistant bacteria.29 If the affected individuals had had the same type of infection but with an antimicrobial-susceptible pathogen, they would have survived.
Mycobacteria, Retroviruses, and Fungi
Typical bacteria like streptococci or Escheria coli are neither the only pathogens to be targeted by antimicrobials nor the only ones able to evade those antimicrobials through development of resistance. In fact, the cycle of discovery and resistance has occurred and continues in every area of infectious disease medicine, driven by microbial evolution, human behavior, and market forces that dictate drug development and dissemination.
The first effective anti-tuberculosis drug, streptomycin, was discovered shortly after penicillin. Selman Waksman won a Nobel Prize for systematic research into antimicrobials produced by soil bacteria, culminating in the discovery of streptomycin.30 This history is complicated by the conflicting perspective of graduate student Albert Schatz, who made significant contributions to the discovery of streptomycin but had been persuaded to sign away his rights to patents or royalties before suing to have these restored.31
The next chapter in anti-tuberculosis drug discovery is perhaps more collegial. In 1951, 3 drug companies reported the almost simultaneous discovery that the compound isoniazid was able to kill Mycobacterium tuberculosis.32 None of these companies would receive a patent for their “new” drug, however. Two doctoral candidates in Czechoslovakia (now the Czech Republic) had already published, back in 1912, a method for producing isoniazid as an example of organic chemical synthesis, completely unaware that it would become a cornerstone of tuberculosis treatment. With industry researchers unable to lay claim to the drug as a “novel invention,” multiple companies took up production of isoniazid, resulting in lower costs and easier dissemination.33
But strains of Mycobacterium tuberculosis quickly developed resistance to either drug, streptomycin or isoniazid, when used alone. Even when used in combination with each other and with other anti-tuberculosis medications, Mycobacterium tuberculosis has proved a challenging target. When researchers began studying drug-resistant tuberculosis, they hypothesized that resistance mutations would make the mycobacterium less fit and less able to spread, limiting drug resistance to treatment-experienced patients.34 But they soon discovered that drug-resistant tuberculosis not only develops in patients receiving partial or sporadic tuberculosis treatment but also spreads, resistance intact, to others. In 2012, nearly 4% of new tuberculosis infections were already resistant to isoniazid and rifampicin, the 2 most important drugs for treatment.35 The rate is as high as 20% in Eastern Europe and Central Asia, while the rates may be underestimated in parts of Africa and the Middle East due to missing data and barriers to comprehensive laboratory assessment for drug-resistant tuberculosis.35
Person-to-person spread of drug-resistant tuberculosis was first described in the context of another infectious disease, human immunodeficiency virus (HIV). In the early 1990s in New York City, public health officials observed an increase in the prevalence of drug-resistant tuberculosis, particularly in patients with HIV, even in the absence of prior treatment.36 They hypothesized that close networks of patients with overlapping risk factors for HIV and tuberculosis, as well as impaired immune function leading to more severe, rapidly progressive tuberculosis infections, might explain these findings.36
The first antiretroviral drug to treat HIV—zidovudine, or AZT—was approved in 1987.37 Treatment with AZT provided little reprieve in the HIV epidemic, however, because the same story of antimicrobial resistance played out for antiretrovirals as had for anti-tuberculosis drugs: first discovery, then resistance, then discovery again, leading to combination therapy. Durable suppression of HIV became possible with the use of triple-drug therapy after 1995, and newer options continue to offer durable suppression with fewer side effects and simpler regimens adapted to individual patients’ needs and preferences. But despite a growing number of options and a greatly improved understanding of HIV viral dynamics, drug resistance remains a challenge for many patients who lived through the early days of less effective antiretroviral therapies or for patients who have experienced sporadic or incomplete treatment.38 As with antibacterial resistance and anti-tuberculosis drug resistance, antiretroviral resistance disproportionately affects people living in LMICs, where HIV is more prevalent and where reliable access to the latest treatments (as well as tools for diagnosis, monitoring, and detecting resistance) depends on global resource sharing as well as economic and infrastructure development.39
As with HIV and tuberculosis, patients with compromised immune systems who deal with more severe or prolonged infections are at increased risk for antimicrobial resistance. Patients with compromised immune systems due to cancer, organ transplantation, HIV, or other conditions bear the greatest burden from serious infections due to fungi. Like humans, fungi are eukaryotes, meaning they rely on similar cellular machinery for survival and growth. For antifungals to be clinically useful, they must target factors unique to fungi and absent in or nonessential to human cells to avoid off-target toxicity in the patient being treated.40 This narrow set of therapeutic targets contributed—and continues to contribute—to the relative lack of antifungals. Emergence of resistance to even one class of antifungals, where alternatives are limited, can have devastating consequences for patients with serious fungal infections.40
A Future of Antimicrobial Resistance
Antimicrobial resistance is a pressing threat to global health. The unifying themes across pathogen types are clear: the discovery of antimicrobials has driven down mortality from infectious diseases, including bacteria, fungi, mycobacteria, and viruses. Pathogens undergo mutations that, with the selective pressure from exposure to antimicrobials, lead to emergence and persistence of antimicrobial resistance. Where the burden of infection is greatest—whether because of compromised immunity or geopolitical forces—and where access to antimicrobials is inconsistent or unstable, resistance thrives. As a result, our victories against death from infectious diseases are inequitably distributed and tenuous.
Beyond the ethical and moral imperatives to reduce suffering and disease, the COVID-19 pandemic has illustrated that infectious threats anywhere are infectious threats everywhere and that one threat (a virus) can have downstream implications for a wide range of infectious diseases and their treatments.41 Colonial-era mentalities regarding borders and the segregation of illness and poverty are incongruent with the reality of antimicrobial resistance as a global health threat. Preserving past successes and advancing our battle against infectious diseases requires continued discovery, novel therapeutics, improved global health infrastructure, and robust collaborations among stakeholders in the antimicrobial development process. We must act now to ensure that the wonder drugs of yesteryear remain viable options for treating the patients of today and to ensure that the wonder drugs of tomorrow will be available worldwide, wherever they are needed most.
References
- Fleming A. On the antibacterial action of cultures of a penicillium, with special reference to their use in the isolation of B. influenzae. Br J Exp Pathol. 1929;10(3):226-236.
- Darby EM, Trampari E, Siasat P, et al. Molecular mechanisms of antibiotic resistance revisited. Nat Rev Microbiol. 2023;21(5):280-295.
- Smith WPJ, Wucher BR, Nadell CD, Foster KR. Bacterial defences: mechanisms, evolution and antimicrobial resistance. Nat Rev Microbiol. 2023;21(8):519-534.
- Ehrlich P. Experimental researches on specific therapeutics. The Harben Lectures. J R Inst Public Health. 1907;15(6):321-340.
- Craddock S. Use of penicillin in cultivation of the acne bacillus. Lancet. 1942;239(6193):558-559.
- Chain E, Florey HW, Gardner AD, et al. Penicillin as a chemotherapeutic agent. Lancet. 1940;236(6104):226-228.
- Abraham EP, Chain E, Fletcher CM, et al. Further observations on penicillin. Lancet. 1941;238(6155):177-189.
-
Abraham EP, Chain E. An enzyme from bacteria able to destroy penicillin. Nature. 1940;146(3713):837.
- Demerec M. Origin of bacterial resistance to antibiotics. J Bacteriol. 1948;56(1):63-74.
- Ventola CL. The antibiotic resistance crisis: part 1: causes and threats. P T. 2015;40(4):277-283.
- Colebrook L, Kenny M. Treatment of human puerperal infections, and of experimental infections in mice, with prontosil. Lancet. 1936;227(5884):1279-1281.
-
Little JS, Dedrick RM, Freeman KG, et al. Bacteriophage treatment of disseminated cutaneous Mycobacterium chelonae infection. Nat Commun. 2022;13(1):2313.
- Schooley RT, Biswas B, Gill JJ, et al. Development and use of personalized bacteriophage-based therapeutic cocktails to treat a patient with a disseminated resistant Acinetobacter baumannii infection. Antimicrob Agents Chemother. 2017;61(10):e00954-e17.
- Barber M. Methicillin-resistant staphylococci. J Clin Pathol. 1961;14(4):385-393.
- Knothe H, Shah P, Krcmery V, Antal M, Mitsuhashi S. Transferable resistance to cefotaxime, cefoxitin, cefamandole and cefuroxime in clinical isolates of Klebsiella pneumoniae and Serratia marcescens. Infection. 1983;11(6):315-317.
- Leclercq R, Derlot E, Duval J, Courvalin P. Plasmid-mediated resistance to vancomycin and teicoplanin in Enterococcus faecium. N Engl J Med. 1988;319(3):157-161.
- Yigit H, Queenan AM, Anderson GJ, et al. Novel carbapenem-hydrolyzing β-lactamase, KPC-1, from a carbapenem-resistant strain of Klebsiella pneumoniae. Antimicrob Agents Chemother. 2001;45(4):1151-1161.
-
Fenton KA, Ison C, Johnson AP, et al; GRASP collaboration. Ciprofloxacin resistance in Neisseria gonorrhoeae in England and Wales in 2002. Lancet. 2003;361(9372):1867-1869.
- Mangili A, Bica I, Snydman DR, Hamer DH. Daptomycin-resistant, methicillin-resistant Staphylococcus aureus bacteremia. Clin Infect Dis. 2005;40(7):1058-1060.
- Humphries RM, Yang S, Hemarajata P, et al. First report of ceftazidime-avibactam resistance in a KPC-3-expressing Klebsiella pneumoniae isolate. Antimicrob Agents Chemother. 2015;59(10):6605-6607.
- Lockhart SR, Etienne KA, Vallabhaneni S, et al. Simultaneous emergence of multidrug-resistant Candida auris on 3 continents confirmed by whole-genome sequencing and epidemiological analyses. Clin Infect Dis. 2017;64(2):134-140.
- Moore PR, Evenson A, Luckey TD, McCoy E, Elvehjem CA, Hart EB. Use of sulfasuxidine, streptothricin, and streptomycin in nutritional studies with the chick. J Biol Chem. 1946;165(2):437-441.
-
Kirchhelle C. Pharming animals: a global history of antibiotics in food production (1935-2017). Palgrave Commun. 2018;4:96.
- Levy SB, FitzGerald GB, Macone AB. Spread of antibiotic-resistant plasmids from chicken to chicken and from chicken to man. Nature. 1976;260(5546):40-42.
-
Center for Veterinary Medicine. 2021 Summary report on antimicrobials sold or distributed for use in food-producing animals. US Food and Drug Administration; 2022. Accessed November 15, 2023. https://www.fda.gov/media/163739/download?attachment
- Gwynn MN, Portnoy A, Rittenhouse SF, Payne DJ. Challenges of antibacterial discovery revisited. Ann N Y Acad Sci. 2010;1213(1):5-19.
- McAdams D. Resistance diagnosis and the changing economics of antibiotic discovery. Ann N Y Acad Sci. 2017;1388(1):18-25.
- Laxminarayan R, Matsoso P, Pant S, et al. Access to effective antimicrobials: a worldwide challenge. Lancet. 2016;387(10014):168-175.
-
Murray CJL, Ikuta KS, Sharara F, et al; Antimicrobial Resistance Collaborators. Global burden of bacterial antimicrobial resistance in 2019: a systematic analysis. Lancet. 2022;399(10325):629-655.
-
Woodruff HB. Selman A. Waksman, winner of the 1952 Nobel Prize for physiology or medicine. Appl Environ Microbiol. 2014;80(1):2-8.
- Wainwright M. Streptomycin: discovery and resultant controversy. Hist Philos Life Sci. 1991;13(1):97-124.
- Murray JF, Schraufnagel DE, Hopewell PC. Treatment of tuberculosis. A historical perspective. Ann Am Thorac Soc. 2015;12(12):1749-1759.
-
Londeix P, Frick M. Isoniazid/rifapentine (3HP) access roadmap and patent landscape. Treatment Action Group; 2020. Accessed November 11, 2023. https://www.treatmentactiongroup.org/wp-content/uploads/2020/03/3hp_access_roadmap_and_patent_landscape.pdf
- Vilchèze C, Jacobs WR Jr. The isoniazid paradigm of killing, resistance, and persistence in Mycobacterium tuberculosis. J Mol Biol. 2019;431(18):3450-3461.
-
Seung KJ, Keshavjee S, Rich ML. Multidrug-resistant tuberculosis and extensively drug-resistant tuberculosis. Cold Spring Harb Perspect Med. 2015;5(9):a017863.
- Frieden TR, Sterling T, Pablos-Mendez A, Kilburn JO, Cauthen GM, Dooley SW. The emergence of drug-resistant tuberculosis in New York City. N Engl J Med. 1993;328(8):521-526.
- De Clercq E. The history of antiretrovirals: key discoveries over the past 25 years. Rev Med Virol. 2009;19(5):287-299.
-
Antiretroviral drug discovery and development. National Institute of Allergy and Infectious Diseases. November 26, 2018. Accessed November 11, 2023. https://www.niaid.nih.gov/diseases-conditions/antiretroviral-drug-development
-
Fact sheet: HIV drug resistance. World Health Organization. November 17, 2022. Accessed November 11, 2023. https://www.who.int/news-room/fact-sheets/detail/hiv-drug-resistance
- Perlin DS, Rautemaa-Richardson R, Alastruey-Izquierdo A. The global problem of antifungal resistance: prevalence, mechanisms, and management. Lancet Infect Dis. 2017;17(12):e383-e392.
-
Kates O. Ethics Talk: antibiotic stewardship during COVID. AMA Journal of Ethics. May 17, 2021. Accessed November 15, 2023. https://journalofethics.ama-assn.org/videocast/ethics-talk-antibiotic-stewardship-during-covid